Background
Many cultural heritage and historic collections house objects that require insect eradication or long-term anoxic (low-oxygen) storage. Optimal anoxic treatments involve placing the object(s) in sealed, oxygen-impermeable enclosures, reducing the oxygen content to less than 1%, and maintaining the anoxic environment for a given period (Shashoua 1999; Hacke et al. 2014; Shashoua 2014; Trafford 2017). Insect eradication can take up to four weeks1 of anoxia, while anoxic storage can last the lifetime of the object (Selwitz et al. 2010: 50; Gulotta 2015:929; Integrated Pest Management Working Group 2020; Cnossen 2021).
To establish an anoxic environment, preservationists decrease oxygen concentrations by purging with nitrogen or argon gas2 or using a chemical that binds with oxygen (called an “oxygen scavenger”) such as AGELESS®. The anoxic environment is maintained with a regular flow of the purging gas, as needed, or by including enough oxygen scavenger to account for potential leakage (Shashoua 1999:886).
This case study builds upon research initiated by the Robert Rauschenberg Foundation (RRF) concerning the cost and environmental feasibility of anoxia for sensitive materials housed within their collection.3 The case study sculpture, Untitled (Venetian), 1973 is one of many pieces in Rauschenberg’s Venetian series that contains oxygen-sensitive materials (Figure 1). The sculpture measures 218 centimeters high by 213 centimeters wide and 41 centimeters deep. It is comprised of plywood, canvas, cardboard, wood glue, and automobile tire rubber. Both the cardboard box and the tire rubber, which exhibit surface cracking, are oxygen sensitive materials that might benefit from anoxic storage to slow the degradation process (Shashoua 1999; Hacke et al. 2014; Dyer et al. 2011). Anoxic storage is one solution for this particular work. Any approach to preservation must consider the artist’s documented attitude toward natural deterioration of his artworks, and possible replacement of deteriorated components. Rauschenberg’s history of prioritizing environmental concerns should also be considered when choosing long term storage due to the resources necessary to maintain a low oxygen environment. The three anoxic methods studied in this report are among many available to practitioners, but a literature review, personal communications, and a survey of practitioners indicate that they are often preferred due to human health and safety, availability, cost, and effectiveness.
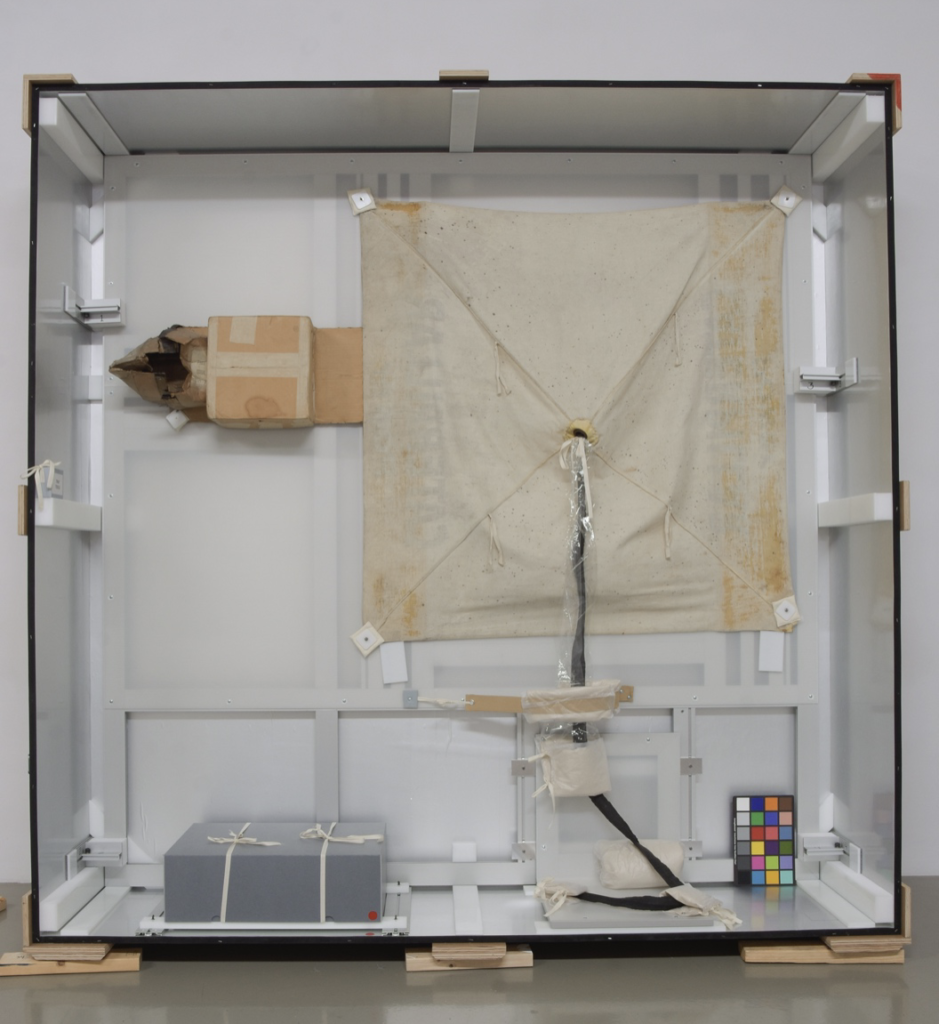
Photograph courtesy Rober Rauschenberg Foundation.
Storing an object for decades in an anoxic chamber requires significant amounts of oxygen-replacing gas or oxygen scavengers. The size of the case study sculpture also creates a challenge for long-term storage, as larger enclosures have more seams and higher air volumes, increasing the chance of leaks and the potential time, materials, and labor required for maintenance (Liebhold 2011). Insect eradication requires the same materials, though for shorter periods of time and therefore use smaller quantities.
Manufacturing, delivering, using, and disposing of the materials needed for anoxic treatments has environmental implications, and it has been unclear which treatment method is environmentally preferable. This life cycle assessment (LCA) case study aims to provide preservationists with information on the relative environmental performance of anoxic systems for pest eradication and long-term preservation of oxygen sensitive materials. The results of this study will help RRF and other preservationists make environment-informed choices between anoxic treatments, and decide whether establishing and maintaining an anoxic enclosure is worth the environmental impact, beyond the cost and time commitments.
1 Maekawa et al. suggests 14-22 days for most pest eradication (2003, 10), however, personal conversations and a survey (Cnossen 2021) indicate that 4 weeks is the standard set by many professionals performing anoxic treatment in order to ensure that the pest eradication is successful.
2 Carbon dioxide is another method for anoxia but was excluded from this case study due to the high human health risk (Maekawa 2003, 8).
3 Other notable uses of anoxia for cultural heritage include the Charters of Freedom —the Declaration of Independence, the Constitution and the Bill of Rights— and the first map labeling the “New World,” as “America” (National Institute of Standards and Technology 2007; United States Department of Commerce 1951).
LCA Modeling
Life cycle assessment (LCA) is an internationally standardized (ISO 14040) modeling tool commonly used to quantify environmental and health impacts of a product or process (ISO 14040:2006). LCA takes a holistic, systems approach by considering sustainability over the entire product life cycle, including raw materials extraction, manufacturing, transport, use, and end-of-life (Vigon et al. 2020; Fava and Society of Environmental Toxicology 1991; United Nations Environment Programme 2005). LCA has been implemented across all types of sectors to inform design and procurement decisions, and provides the scientific basis for all carbon, water, and environmental footprinting. For example, popular online calculators use LCA to compare different grades of paper or packaging materials (Environmental Paper Network 2021; sphera 2020).
For this case study, LCA modeling was completed using the free, open-source software OpenLCA (v1.10.3) with material-specific data from the ecoinvent database (v3.7, APOS) (ecoinvent 2020; GreenDelta 2020). Global warming (or carbon footprint, measured in carbon dioxide equivalents) and nine other categories of environmental and human health impacts for each scenario were calculated using the Tool for the Reduction and Assessment of Chemical and other environmental Impacts (TRACI v2.1) impact assessment method developed by the U.S. Environmental Protection Agency (U.S. EPA 2012). Further information concerning tools and methods can be found in the STiCH Introduction to LCA Information Sheet.
This case study covered a ‘cradle-to-grave’ scope, examining all life cycle stages of the products used to fulfill each scenario (Figure 2). LCA compares options based on the function that they provide – called a ‘functional unit’- ensuring fair comparisons. The functional unit for this case study was an anoxic treatment volume of 3,060,000 cm3 (3.06 m3, ~108 ft3).
Methods
This case study models three common low-oxygen systems: AGELESS® ZPT oxygen absorber, nitrogen gas, and argon gas. Each anoxic scenario was applied to three time periods in order to represent a variety of preservation situations: a four week pest eradication treatment, a one year long-term storage, and a five year long-term storage. The enclosure was based on the size of the storage box built for Untitled (Venetian), 1973 and material quantities were based on technical guidance and practitioner experience. All methods to achieve anoxia generally increase in complexity and cost as the enclosure size increases (Wolfe et al. 2001: 80).
AGELESS® is typically used to maintain anoxia in small enclosures for short-term pest eradication but has also been used for long-term anoxic storage (Dyer et al. 2011; Shashoua 1999; Gilberg et al. 1994). For large enclosures AGELESS® is usually considered impractical as a long-term storage solution (Maekawa 2003:19), although it is sometimes a viable option.
MODELING PROCESS
Professionals typically build and maintain anoxic chambers from standard materials, so the scope included the items determined to be most necessary and common (Cnossen 2021). This study modeled a typical enclosure largely based on materials specified by Maekawa (2003). The enclosure interior measures 234 cm x 239 cm x 54 cm, which equals a total volume of 3,060,000 cm3 (~3.06 m3). Materials modeled in this study were divided into four categories: Enclosure, Gas Set-Up, Gas Treatment, and AGELESS®.
Depending on the length of treatment, some items may need to be replaced. Cultural heritage preservationists may vaguely reference the “lifetime” of a material or product used for treatment without a specific period in mind (10 years, 20 years, more?). However, LCA modeling requires a definitive use period. For this case study, lifetimes of various components required for treatment were estimated either according to product warranties or approximated use periods based on conversations with practitioners.
The LCA Modeling sub-sections describe the materials modeled for each category and their respective lifetimes; Table 1 summarizes this information.
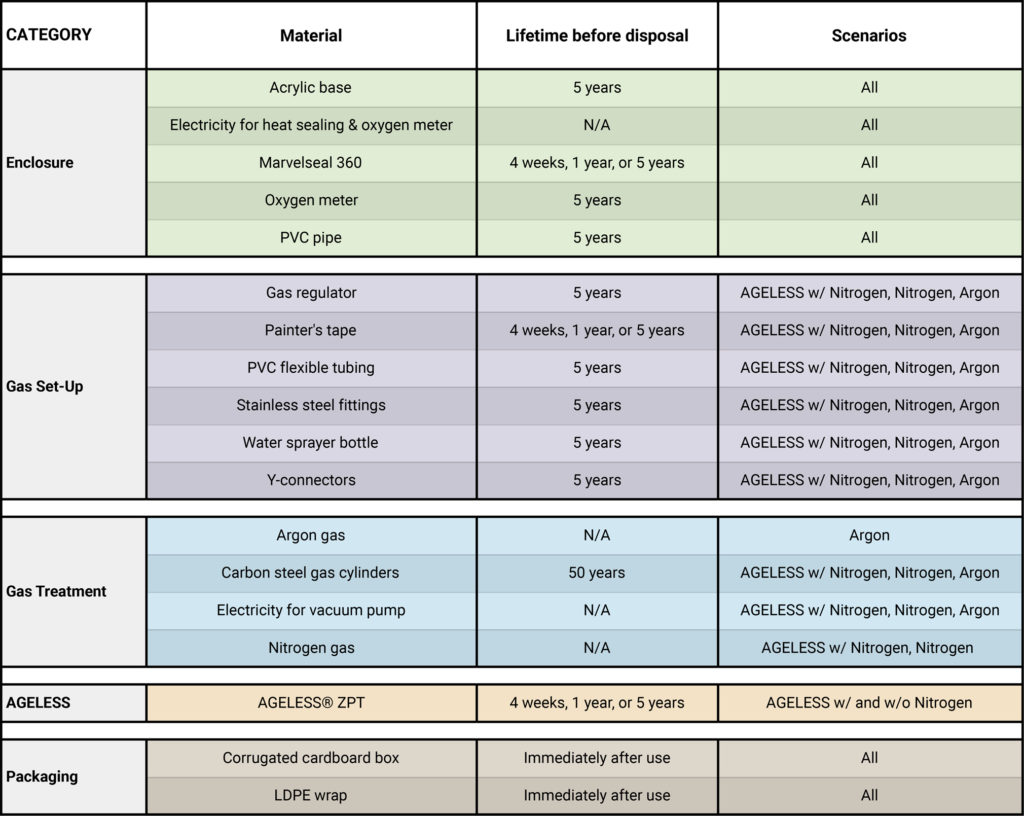
ENCLOSURE
The enclosure includes: an acrylic base to protect the enclosure sheet floor, polyvinyl chloride (PVC) pipe for the frame, Marvelseal 360 (layers of nylon, polyethylene, aluminum foil, and linear low-density polyethylene) to cover the frame and create an air-tight space, and an oxygen meter to measure the oxygen level within. The calculations also include the electricity needed to heat-seal the Marvelseal 360 seams and to power the oxygen meter.4 The acrylic base, PVC pipes, and oxygen meter were assumed to have a five year lifetime before disposal. The impacts of manufacturing these three products are thus attributed according to each treatment or storage length. For example, one year of chamber use would account for one-fifth of the total environmental impacts from the acrylic base, PVC pipes, and the oxygen meter.
GAS SET-UP
The gas set-up was modeled as flexible tubing (PVC), Y-connectors (polypropylene), stainless steel fittings (valves, barbed regulator adapters, plugs), a gas regulator (brass and glass), a 3-gallon plastic water sprayer bottle, and painter’s tape. The PVC flexible tubing, polypropylene y-connectors, stainless steel fittings, and water sprayer bottle are assumed to have a five year lifetime. The painter’s tape is assumed to last the full length of each treatment or storage without replacement.
GAS TREATMENT
Nitrogen and argon are inert gasses commonly used to create anoxic environments in the cultural heritage field. There is evidence that argon can be a better eradicator of some pests than nitrogen (Valentin 1993), but literature also supports the use of nitrogen (Selwitz et al. 2010; Daniel et al. 1993; Maekawa et al. 2003). The gas treatment was modeled as the quantity of nitrogen or argon needed for each treatment scenario and the electricity needed to operate a vacuum pump.5 All gas treatments consist of 48-hour purges and the frequency of the purges varies depending on the treatment type and length. The amount of gas needed for each 48-hour purge is eight times the enclosure volume (Maekawa 2003:108).6
Inputs and materials for producing the 50-liter carbon steel gas cylinders were also included. The cylinders were assumed to have a 50 year lifetime and the impacts of manufacturing the cylinders were attributed based on the treatment or storage scenario duration.
AGELESS®
AGELESS® ZPT was chosen for this study because it is functionally the closest oxygen absorber to AGELESS® Z, which is no longer available but the most commonly mentioned in literature (Shashoua 1999; Dyer et al. 2011; Carrió et al. 2002; Gilberg et al. 1994; Shashoua et al. 1993). Mitsubishi Gas Company defines AGELESS® ZPT as a standard self-reacting iron-based oxygen absorber for medium to high moisture-containing products (Japan Mitsubishi Gas Chemical Company, INC., 2011). AGELESS® was modeled as a mixture of diatomaceous earth (bentonite was selected as the closest available proxy) and iron powder based on information from safety data sheets and retailers. The packets that store the AGELESS® were modeled as sack kraft paper, plastic liners, and glues. The 3.06 m3 enclosure required 307 packets of AGELESS® ZPT-3000 to attain less than 1% oxygen concentration. 7,8
Product packaging for all components (except for the gas cylinders) was assumed to be one layer of low-density polyethylene (LDPE) wrap and a standard corrugated cardboard box to protect the material during transport from the manufacturer to the user.
TRANSPORTATION
Transportation of materials from the manufacturing or retail site to the treatment site was modeled as 1,000 kilometers by truck, a standard assumption in LCA modeling to represent average shipping distances. Transportation of the gas cylinders was modeled as 80 kilometers by truck each way, as the full cylinder is transported from the manufacturer to the user and then the empty cylinder must also be transported from the user back to the manufacturer to be refilled and reused.
DISPOSAL
All enclosure materials and the gas set-up components were assumed to last the entire treatment length without replacement. All materials required to assemble the enclosure, set-up for the gas, the AGELESS®, and the packaging were assumed to be incinerated as standard municipal solid waste without energy recovery at the end of their respective lifetimes. The exception is the stainless steel fittings used in the gas set-up; these components are assumed to be recycled at the end of their assumed five year lifetime.
STUDY SCOPE
Figure 2 shows the overall system diagram of the study, including the various inputs, processes, and scenarios. This study is focused on the treatment process and so excluded the materials and inputs needed to produce the original artwork Untitled (Venetian), 1973. Silica gel or other inputs needed to control humidity were also excluded to keep the focus on the methods for removing the oxygen, not on maintaining other aspects of the environment. Humidity control is more variable than anoxic treatment and would require a separate study.
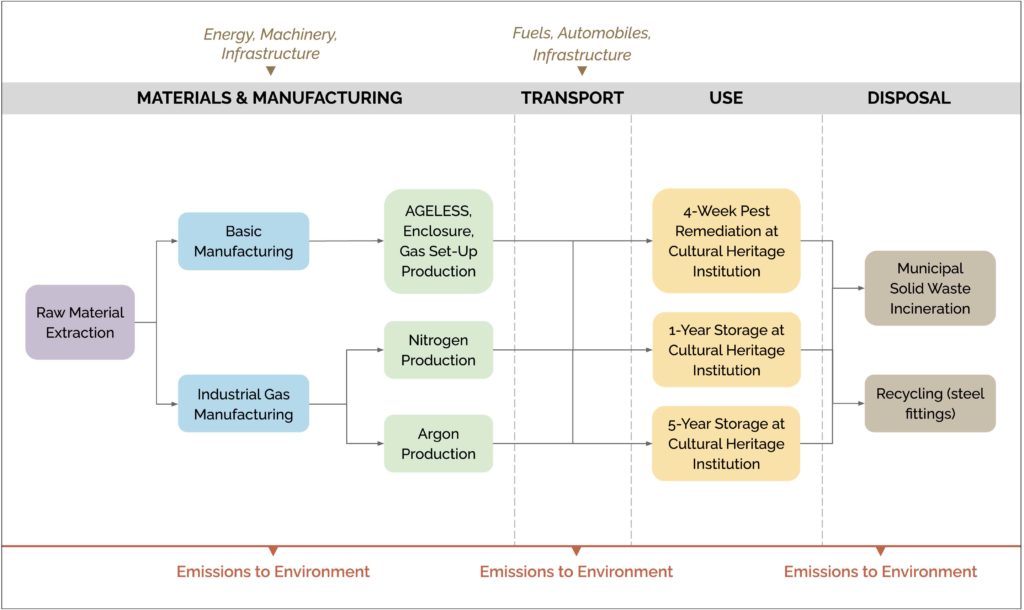
4 In order to calculate the amount of electricity the oxygen monitor uses during each scenario, a number of assumptions had to be made. Based on manufacturer specifications, it was assumed that the oxygen monitor has a wattage of 0.5 watts. Additionally, it was assumed that the monitor was running for two hours every workday and for a continuous 48 hours for every purge throughout the duration of the treatment or storage period.
5 The vacuum pump evacuates air throughout the 48-hour nitrogen or argon purges (Maekawa 2003).
6 For nitrogen gas: (3060485 / 1000) x 8 x (1 / 24.5) x (28 / 1000) = 28 kg N per 48-hour purge
For argon gas: (3060485 / 1000) x 8 x (1 / 24.5) x (40 / 1000) = 40 kg Ar per 48-hour purge
7 This quantity was determined using the following calculations:
3062.031 liters air x 0.20 (oxygen is 20% of total air volume) = 612.406 liters oxygen to be treated in the enclosure
AGELESS® ZPT-3000 (“the 3000 stands for the ability of one packet to remove absorb 3 liters or 3,000 cc of oxygen”)
612.406 liters oxygen x (1 AGELESS® ZPT-3000 packet / 3 liters oxygen) = 204.135 packets
Approximately 50% should be added for safety to make up for any enclosure leakage
204.135 packets + (0.5 x 204.135) = 306.203 packets → roundup to a whole number
307 packets of Ageless ZPT-3000 are needed to treat the enclosure volume
https://conservationsupportsystems.com/product/show/ageless-zpt-2000/oxygen-absorbers-ageless-zpt
8 Because AGELESS® is not frequently used in enclosures as large as the study prototype, a number of assumptions had to be made. Practical concerns regarding the logistics of placing the 307 individual AGELESS® packets without overlap, were not considered.
Results
Figures 3-5 show the results for each treatment or storage duration. Results for each option are divided into six categories (represented by color blocks) according to the contribution of each life cycle stage or material type. Packaging was totaled for each material type, it is not a separate color block. The LCA Modeling section above goes into further detail about what was included in each of these categories. Tables 2 and 3 explore alternative purge scenarios for the one year and five year storage durations.
Readers should note that the final results shown in Figures 3-5 and Tables 2 and 3 cannot simply be divided or multiplied to calculate the total results for a chamber that is smaller or larger than the study size. Not all of the component quantities are directly proportional to the chamber size, except for the gas treatment (blue color block), which is proportional and also the largest contributor for many of the scenarios.
FOUR WEEK PEST REMEDIATION
The four scenarios considered for the four week pest remediation treatment were AGELESS® without and with one 48-hour nitrogen purge, two 48-hour nitrogen purges, and two 48-hour argon purges, shown in Figure 3. AGELESS® with one nitrogen purge has a 60% larger carbon footprint than AGELESS® without the nitrogen purge. The global warming impact of AGELESS® with one nitrogen purge and the nitrogen treatment (two nitrogen purges with no AGELESS®) came within 7% CO2 eq. of one another. The argon scenario has the largest global warming impact and is more than double the AGELESS® with one nitrogen purge and two nitrogen purges.
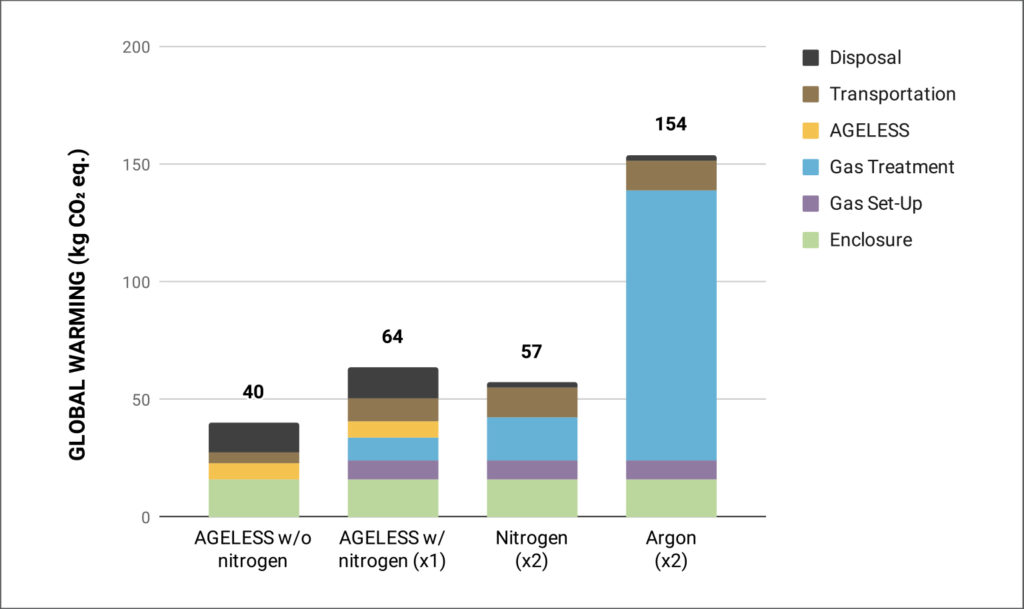
Nitrogen and argon gasses are both manufactured using a process called cryogenic air separation. It takes more energy to separate argon than it does nitrogen because air has a significantly smaller proportion of argon (~0.9%) than nitrogen (78%). This difference in required energy results in argon having a larger carbon footprint intensity (CO2 eq. per kilogram of gas) than nitrogen. These carbon footprint intensities are reflected in the Figure 3 results, where the argon gas treatment (shown in blue) has a global warming impact that is 6.4 times greater than the nitrogen gas treatment.
ONE YEAR ANOXIA STORAGE
The three scenarios for the one year anoxia storage were AGELESS® with one nitrogen purge, nitrogen with twenty-four 48-hour purges, and argon with twenty-four 48-hour purges (two purges per month) shown in Figure 4. The AGELESS® scenario has the lowest global warming impact, with a 78% lower impact than the nitrogen and a 94% lower impact than the argon. The nitrogen has a 74% lower impact than the argon.
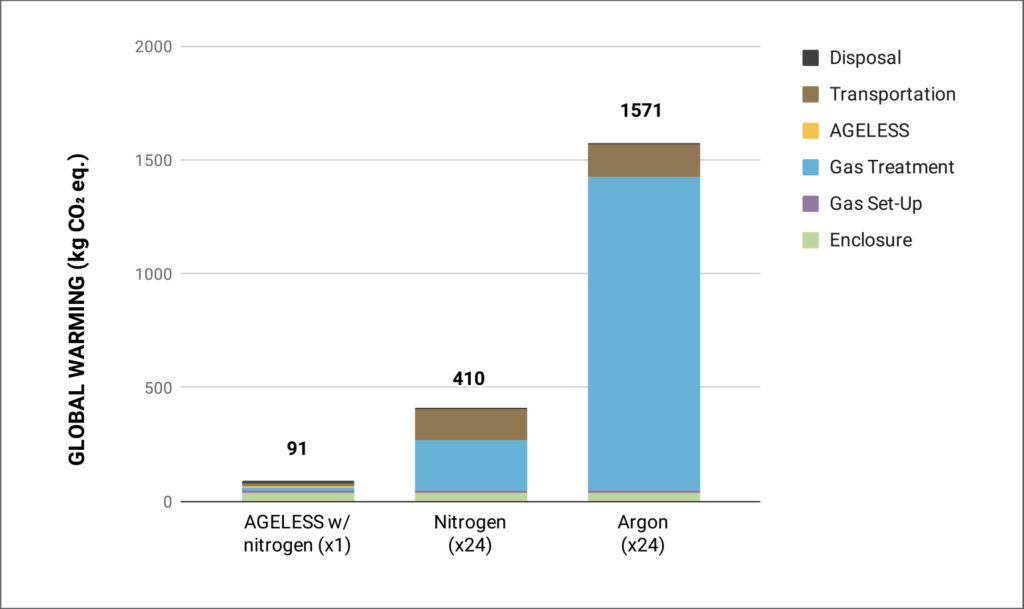
Results for alternative scenarios, increasing and decreasing frequency of nitrogen and argon purges to be weekly or monthly, for the one year treatment are reflected in Table 2 below. Because the gas treatment step is the largest contributor to the two gas purging options, decreasing or increasing the frequency of the gas purges significantly decreases or increases, respectively, the total global warming impact for that scenario.
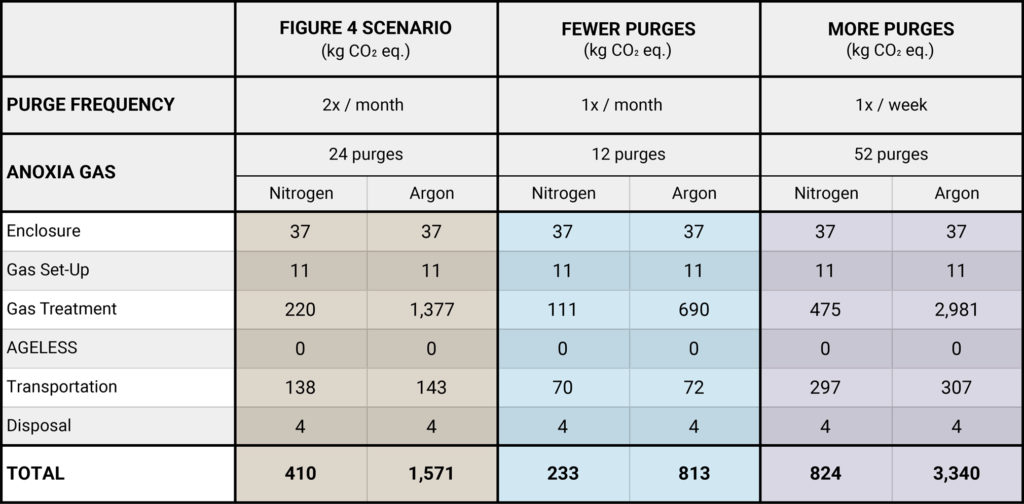
FIVE YEAR ANOXIA STORAGE
The scenarios for the five year anoxia treatment were one AGELESS® treatment with one 48-hour nitrogen purge, two AGELESS® treatments with two 48-hour nitrogen purges, sixty 48-hour nitrogen purges, and sixty 48-hour argon purges (one per month), shown in Figure 5. In the second scenario, doubling the AGELESS® and nitrogen resulted in a 16% increase in kg CO2 eq. While the 60 nitrogen purges resulted in a global warming impact that is 73% less than the 60 argon purges.
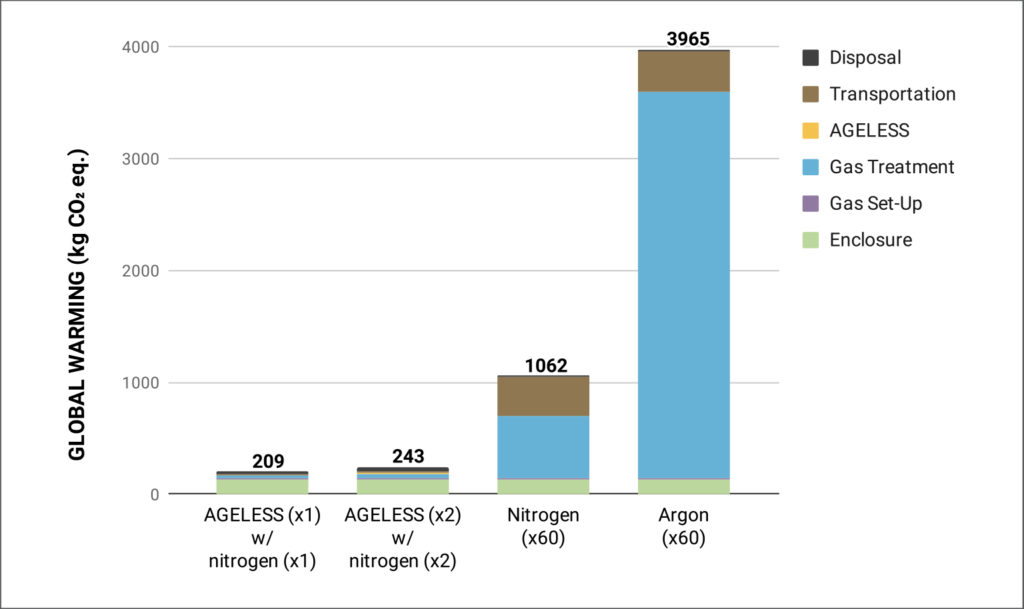
Alternative purging scenarios for the five year treatments are reflected in Table 3 below, which shows a breakdown of the results by category for the nitrogen and argon gas treatments over three different purge frequencies.
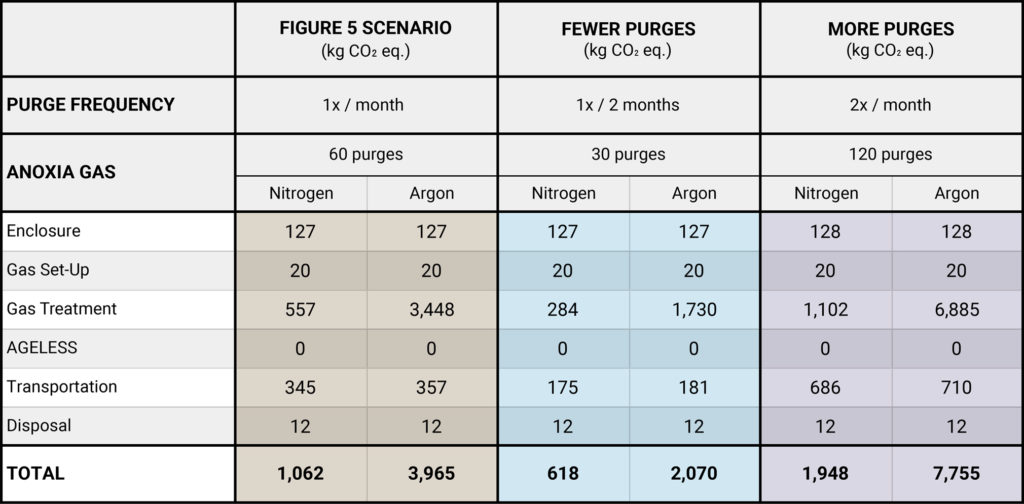
RELATIVE RESULTS FOR FOUR WEEK PEST REMEDIATION
Global warming (measured in CO2 eq.) is just one environmental impact category. Many other environmental and health considerations influence sustainable choices. Figure 6 illustrates the four week pest remediation results across a suite of ten impact categories from the U.S. EPA TRACI method. Each scenario is calculated as a percentage of the scenario with the greatest impact in each category. The argon treatment (in light brown) results in the largest impact compared to the other three scenarios across all impact categories except for ecotoxicity, where AGELESS® with one nitrogen purge has the largest impact. AGELESS® without the nitrogen purge results in the lowest impacts across all of the impact categories except ecotoxicity and non-carcinogenics, indicating that it is likely the most sustainable choice for this treatment scenario.
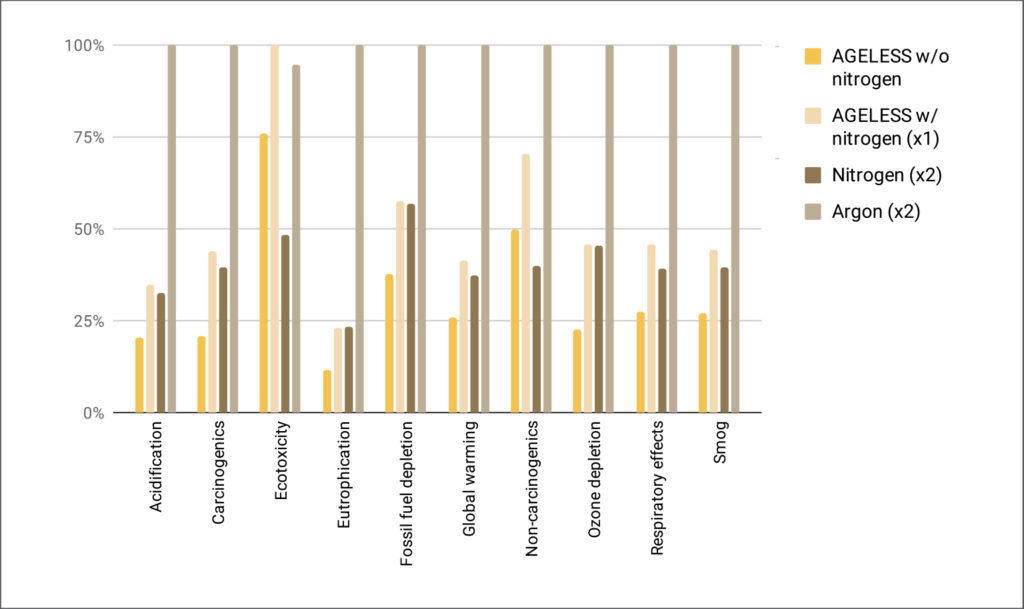
Discussion and Conclusion
Choosing to encapsulate an object in a low oxygen environment involves a continuous commitment of resources including materials, maintenance, and cost. Once an object is encapsulated, removal puts it at risk, so neither decision can be made lightly. This study assigned five years as the length for long-term storage, but in reality the duration would concur with the lifetime of the object. Projecting the environmental impact for long-term storage beyond five years adds more temporal uncertainty into the results due to expected differences in yearly emission inventories. The results for one year of anoxia storage cannot simply be divided or multiplied to calculate the total results for shorter or longer storage times because not all of the component quantities are directly proportional to the storage length.
In all scenarios argon results in the greatest impact, and choosing nitrogen and/or AGELESS® to achieve the anoxic environment, no matter the duration, presents a clear path for lower impact methods. Chamber setups contributed a notable impact, so variations in methods between practitioners will somewhat alter LCA results and reuse is key to saving resources, reducing emissions, and controlling waste. Selecting lower impact materials to construct the chamber can be explored.
Although this study compares one anoxic method to another it does not put the resulting environmental impacts into the larger context of personal or professional annual carbon footprints. This context helps individuals and institutions when they examine the environmental cost of their actions and consider alternatives. The EPA provides a tool that allows individuals to compare estimated or calculated CO2 equivalents with impacts resulting from more well-known actions. For example, an enclosure size appropriate for storing an object the size of Untitled (Venetian), 1973 for five years in a nitrogen environment with one purge per month results in 1,062 kg CO2 eq. (Figure 5). Storing the same object for five years in an argon environment with one purge per month results in 3,965 kg CO2 eq. (Figure 5). The EPA GHG Equivalencies Calculator shows that 1,062 kg CO2 eq. is equivalent to greenhouse gas emissions from driving 2,636 miles and 3,965 kg CO2 eq. is equivalent to driving 9,842 miles in an average gasoline-powered passenger vehicle. Once the practitioner understands these equivalents, they are further equipped to make conscientious decisions and consider at what cost to the environment they carry out the remarkable task of preserving cultural heritage.
PROJECT TEAM
Guest Author: Kris Cnossen
Project Team: Matt Eckelman, Sarah Nunberg, Sarah Sanchez
Acknowledgements: Eric Breitung, Ellen Pearlstein, Robert Rauschenberg Foundation
PROJECT HISTORY
Organized efforts to introduce LCA to U.S. cultural heritage preservation professionals began in 2012 with a series of six LCA case studies conducted as a collaboration between conservators and environmental engineers, in partnership with the MFA Boston. FAIC was awarded a Tier I grant in 2016 and a Tier II grant in 2020 from the National Endowment for the Humanities to expand the case study research and develop a carbon calculator for individual materials under the project Sustainability Tools in Culture Heritage, STiCH,9 available to visitors to the AIC website.10
9 Foundation for Advancement in Conservation (FAIC). 2021. “Sustainability Tools in Cultural Heritage (STiCH).” https://stich.culturalheritage.org/.
10 American Institute for Conservation (AIC). 2021. Cultural Heritage. https://www.culturalheritage.org/.
References
Carrió, Vicen, and Suzie Stevenson. 2003. “Assessment of Materials Used for Anoxic Microenvironments.” In Conservation Science 2002: Papers from the Conference Held in Edinburgh, Scotland, 22-24 May 2002, edited by Katherine Eremin, Joyce Townsend, and Annemie Adriaens, 32–38. London : Archetype.
Cnossen, Kris. 2021. Unpublished survey. “Anoxia use in the cultural heritage field” Google Form. Posted in Global Conservation Forum (ConsDistList).
Daniel, Vinod, Gordon Hanlon, and Shin Maekawa. 1993. “Eradication of Insect Pests in Museums Using Nitrogen.” Western Association for Art Conservation Newsletter 15 (3): 15–19.
Dyer, Joanne, Clare Ward, Nicole Rode, Marei Hacke, Yvonne Shashoua, and Kongens Lyngby. 2011. “Reassessment of Anoxic Storage of Ethnographic Rubber Objects.” In ICOM-CC 16th Triennial Meeting Preprints, Lisbon, 19–23 September 2011, edited by Janet Bridgland and Catherine Antomarchi, 1–10. Paris : ICOM CC.
/ecoinvent. 2020. “Systems Models in ecoinvent 3: Allocation at the Point of Substitution.”
Accessed August 2, 2022. https://www.ecoinvent.org/database/system-models-in-ecoinvent-3/apos-system-model/allocation-at-the-point-of-substitution.html.
Environmental Paper Network. 2021. “Paper Calculator: presented by environmental paper
network.” Accessed August 2, 2022. https://c.environmentalpaper.org/.
Fava, James A., and Society of Environmental Toxicology and Chemistry. 1991. A technical framework for life-cycle assessment: workshop report. August 18-23, 1990. N.p.: SETAC.
Gilberg, Mark, and David Grattan. 1994. “Oxygen-Free Storage Using Ageless Oxygen Absorber.” Studies in Conservation 39: 177–180. https://doi.org/10.1179/sic.1994.39.Supplement-2.177.
GreenDelta. 2020. “openLCA.” Accessed August 2, 2022. https://www.openlca.org/.
Gulotta, Davide, Paola Fermo, Lucia Toniolo, and Sara Goidanich. 2015. “Anoxic Treatment for the Disinfestation of Wood Cultural Heritage: Assessment of the Effects and Harmfulness on Different Species.” Wood Science and Technology : Journal of the International Academy of Wood Science 49 (5): 925–44. https://doi.org/10.1007/s00226-015-0748-2.
Hacke, Marei, Jo Willey, Gemma Mitchell, Iain D. Rushworth, Catherine Higgitt, and Lorraine T. Gibson. 2014. “Investigation of Long Term Storage Solutions for Rubber Garments.” Journal of the Institute of Conservation 37 (2): 179–96. https://doi.org/10.1080/19455224.2014.931872.
International Organization for Standardization. 2006. Environmental management – Life cycle assessment – Requirements and guidelines. 2006-07-01 ed. International Standard, ISO 14044. Geneva: ISO.https://www.iso.org/standard/38498.html.
Japan Mitsubishi Gas Chemical Company, INC. 2011. AGELESS® Brochure. http://ageless.mgc-a.com/AGELESS%20brochure.pdf.
Liebhold, Andrew, and Barbara Bentz. 2011. “Insect Disturbance and Climate Change.” Forest Service, Climate Change Resource Center. https://www.fs.usda.gov/ccrc/topics/insect-disturbance-and-climate-change.
Maekawa, Shin and Kerstin Elert. 2003. “The Use of Oxygen-Free Environments in the Control of Museum Insect Pests.” Tools For Conservation. The Getty Conservation Institute, Los Angeles.
Museumpests.Net: Integrated Pest Management Working Group. 2020. “Solutions-Nitrogen/Argon Gas Treatment.” Accessed August 1, 2022. https://museumpests.net/solutions-nitrogenargon-gas-treatment/.
National Institute of Standards and Technology. 2007. “NIST Encasement Now Protecting ‘America’s Birth Certificate.’” Accessed August 2, 2022.
https://www.nist.gov/news-events/news/2007/12/nist-encasement-now-protecting-americas-birth-certificate.
Selwitz, Charles, and Shin Maekawa. 2010. Inert Gases in the Control of Museum Insect Pests – Research in Conservation.
Shashoua, Yvonne, and Scott Thomsen. 1993. “A Field Trial for the Use of Ageless in the Preservation of Rubber in Museum Collections.” In Saving the Twentieth Century: The Conservation of Modern Materials, 363–72.
Shashoua, Yvonne. 1999. “Ageless Oxygen Absorber: From Theory to Practice.” Modern Materials II: 881–87.
Shashoua, Yvonne. 2014. “A Safe Place; Storage Strategies for Plastics.” Conservation Perspectives; Conservation of Plastics, 1–6.
sphera. 2020. “GaBi Packaging Calculator – Sustainable Packaging Design.” Accessed August 2, 2022.
https://gabi.sphera.com/international/software/gabi-envision/gabi-packaging-calculator/.
Trafford, Amy, Lu Allington-Jones, and The Natural History Museum. 2017. “Construction of Anoxic Microenvironments – Project Airless.” Storage Techniques for Art Science & History Collections. Accessed August 2, 2022.
https://stashc.com/the-publication/environment/construction-of-anoxic-microenvironments-project-airless/.
United Nations Environment Programme. 2005. “Life Cycle Approaches: the Road from Analysis to Practice.” N.p.: UNEP/SETAC, Life Cycle Initiative, Paris.
United States Department of Commerce. National Bureau of Standards. 1951. “Preservation of
the Declaration of Independence and the Constitution of the United States.” National Bureau of Standards Circular 505.
United States Environmental Protection Agency. 2012. “Tool for Reduction and Assessment of
Chemicals and Other Environmental Impacts (TRACI).” https://www.epa.gov/chemical-research/tool-reduction-and-assessment-chemicals-and-other-environmental-impacts-traci.
Valentin, Nieves. 1993. “Comparative Analysis of Insect Control by Nitrogen, Argon and Carbon Dioxide in Museum, Archive and Herbarium Collections.” International Biodeterioration and Biodegradation 32 (4): 263–78. Accessed August 2, 2022. https://doi.org/10.1016/0964-8305(93)90029-2.
Vignon, Bruce W., D. Tolle, B. Cornaby, H. Latham, C. Harrison, T. Boguski, R. G. Hunt, and J.
Sellers. 2020. “Life-cycle assessment: Inventory guidelines and principles.” N.p.: CRC Press.
Wolfe, Julie, and Eleonora Nagy. 2001. “Considerations for the Structural Stabilization of Deteriorated Industrial Rubber.” Objects Specialty Group Postprints 8: 77–96.